Previous Work
Genetic control
Riboswitches
Biochemists have for a long time used protein-based transcriptional regulation to control the expression of genes in bacteria.
The classic example of this sort of regulation is the lac operon, a set of genes involved in E. coli lactose metabolism that are transcribed from the lac promoter.
A homotetramer of the protein lacI (the lac repressor) binds to operator sites in the promoter and prevents promoter recognition by RNA polymerase through supercoiling of the DNA. (1)(2) The addition of an inducer such as IPTG causes the protein to unbind so that the operon genes can be transcribed. In the native E. coli system, an additional control point is also present in the form of catabolite repression i.e. regulation based on the carbon-containing substrates of the cell to bias metabolism towards more favourable carbon sources such as glucose. In the case of the E. coli lac operon this regulation takes place using cAMP and the protein transcriptional activator CRP/CAP.
​
​
​
​
​
​
​
The lac promoter can be encoded on expression plasmids upstream of any gene of interest to regulate it via the concentration of IPTG/lactose and glucose. In E. coli, a prokaryotic model organism, multiple different regulated-promoter systems have been studied including the arabinose-inducible pBAD system which uses the transcription repressor/activator araC. (3)
​
​
​
​
​
​
​
​
​
Protein-promoter systems have the advantages of a long research history, good understanding and cheap chemical inducers.
However, protein-promoter systems are difficult to redesign to suit new applications and new organisms. The function of a protein such as araC, which interacts with RNA polymerase, depends upon its 3D-shape, which is determined by its sequence of amino acids. Whilst protein folding is increasingly well understood, the design of a protein with a specific shape and properties is currently not possible. As RNA polymerase, the structure of which varies between organisms, protein-promoter systems are not easily transferable between organisms.
In order to create a complicated system of genes, for instance a network of many interacting genes to form a biological computer, the pool of characterised inducible promoters is rapidly exhausted so a more adaptable and modular method of regulation is required. (4)
Unlike protein-based transcriptional regulation, which is dependent on the complicated tertiary and quaternary folding of the amino acids of a protein sequence and the interaction of the protein with DNA, control at the level of mRNA structure can be predicted and designed through simpler nucleotide interactions.
In general, stable mRNA structures, such as RNA hairpins, near the 5' end of mRNA obstruct the binding of the ribosome to the ribosome-binding site (RBS). This lowers the rate of translation of the mRNA, producing less of the protein product. (5)
Riboswitches (RNA switches) have great potential for the design of synthetic genetic circuits. Riboswitches are RNA structures within a protein-encoding mRNA that contain aptamers:
sequences that bind to small molecules such as amino acids or metal ions. The binding of the small molecule changes the structure of the riboswitch exposing or hiding the ribosome binding site and thereby controlling gene expression. Other riboswitches operate through RNA self-cleavage (e.g. the GlmS ribozyme) or by attenuation of transcription.
Natural riboswitches are present in bacteria allowing regulation of key metabolic genes. A few rare examples such as the glycine riboswitch shown below display cooperative behaviour:
​
​
​
​
​
​
​
​
​
​
​
​
​
Using RNA aptamers generated by in vitro evolution (SELEX), synthetic riboswitches have been produced that respond to a variety of chemicals. (6)
Yin et al. (7) have made riboswitches at the 5’ end of mRNA that are responsive to the presence of a trigger strand of RNA that anneals to a single stranded region to open a hairpin (a toehold mechanism). Opening this hairpin reveals an RBS located in the hairpin loop. By investigating different sequences they managed to achieve ON/OFF ratios greater than 600. As the sequence of the trigger was not greatly restricted multiple versions can be created and they demonstrated independent regulation of four different fluorescent protein reporters.
The AUG start codon in these toehold switches is located in the stem of the hairpin necessitating a translated linker before the protein of interest.
​
​
​
​
​
​
​
​
​
​
One problem with riboswitches is that the folding of the switch is very context-dependent and can therefore be affected by the sequence of the first bit of the downstream gene. This means that replacing the gene by merely replacing the coding-sequence downstream of the start codon with the new sequence can cause riboswitch misfolding. One inelegant solution would be to keep the first 100 codons or so of the original gene to produce a fusion-protein of the original protein and desired protein. This however would not be possible if the desired protein cannot have a long N-terminal linker.
Folliard et al. designed a "riboattenuator" that adds a second RBS sequence, hidden by a small hairpin, after 150bp of the open reading frame (ORF) of the original riboswitch-controlled gene. It is this RBS that is positioned correctly for the gene of interest. This attenuator is opened by a ribosome traveling on the mRNA from the riboswitch RBS tying the translation of the gene of interest to the translation initiation rate of the riboswitch. (8)
​
​
​
​
​
​
​
​
​
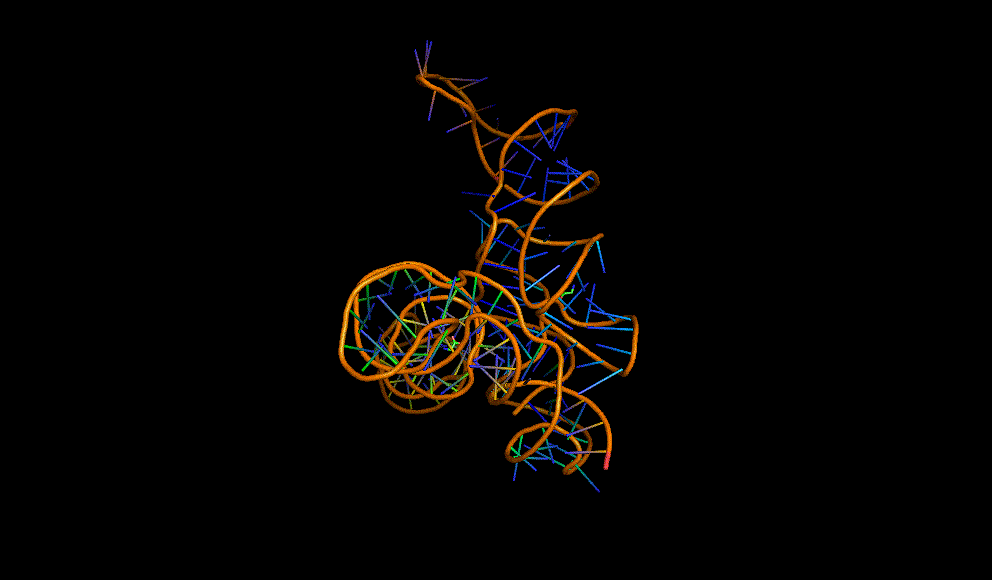
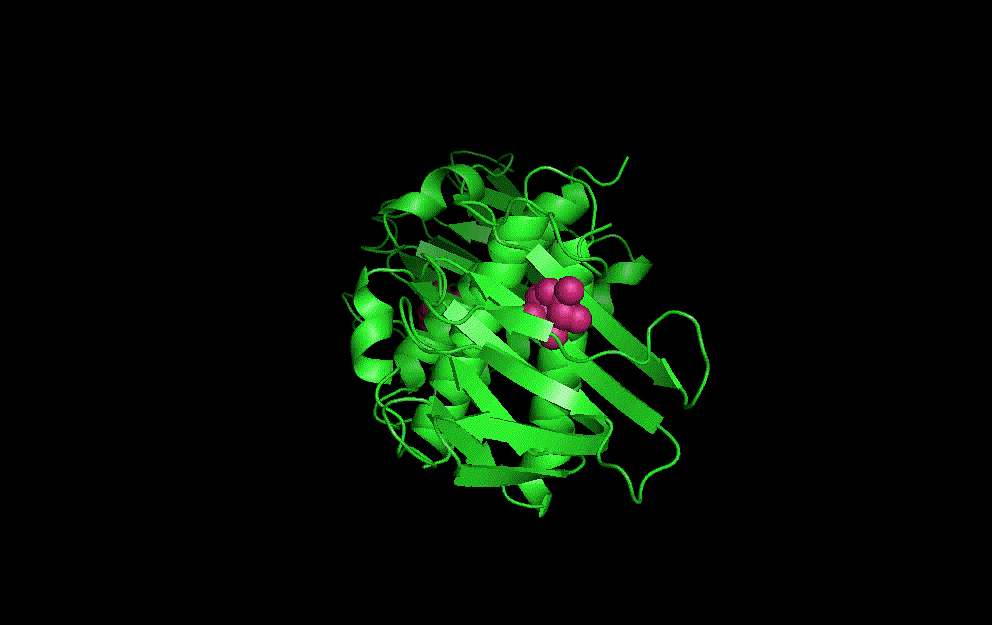
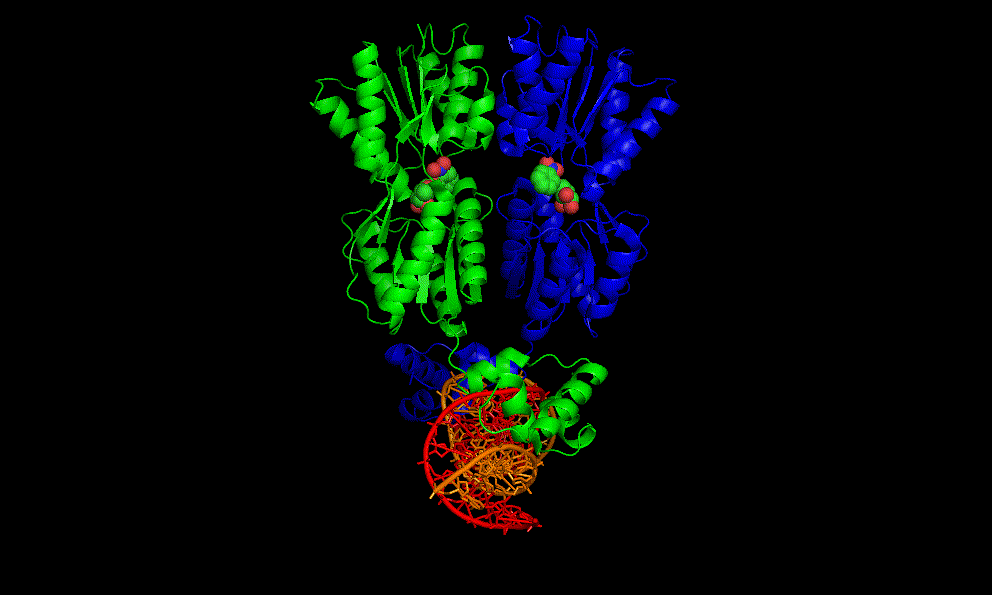
LacI dimer with its operator: 2PE5
The dimerisation and arabinose binding domains of an araC dimer: 2ARC
A cooperative glycine riboswitch from Fusobacterium nucleatum: 3P49 from (16)
Toehold switch template adapted from Yin et al (7)

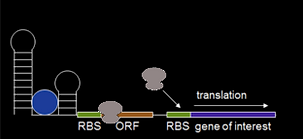
Mechanism of the riboattenuator of Folliard et al. The binding of the ligand (blue) to the riboswitch opens the attenuator using a ribosome translating the first open reading frame. Adapted from (8)
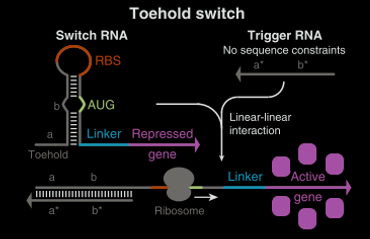
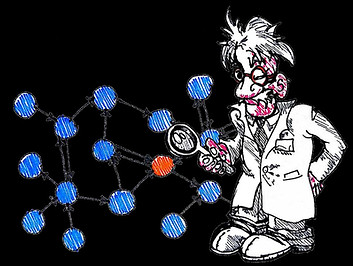
Our riboswitch designs are partly based on cooperative molecular beacons. Molecular beacons are DNA or RNA molecules that form hairpin structures typically with the ends labelled with fluorophore-quencher pairs. The binding of a complementary sequence to the loop region is able to unwind the hairpin to separate the fluorophore-quencher pair leading to a detectable fluorescence output. Molecular beacons can be used for detecting specific DNA or RNA sequences in a sample, for instance the detection of viral RNA.
​
​
​
​
​
​
​
​
​
Plaxco et al (9) created molecular beacons that were cooperative with respect to their trigger concentration i.e. instead of a broad dose-response relationship there was switch-like behaviour.
​
​
​
​
​
​
​
​
Cooperativity in these systems arises because the first trigger binding event is of low affinity, in this case because it requires the unfavourable unwinding of the stem of the hairpin, whilst the second binding event is of greater affinity, as the hairpin stem in already unwound and the complementary bases are exposed.
The degree of cooperativity, quantified by the hill coefficient n, rapidly approaches its maximum (equal to the number of binding sites) as the affinity of the second binding event trends to infinitely more favourable than the first.
Plaxco also generated cooperative molecular receptors, using a different design consisting of two tandem copies of one half of an aptamer, a long unstructured linker, then two copies of the second half of the aptamer. (10)
​
​
​
​
​
​
​
​
​
Cooperativity arises in this design as the first binding event must overcome the unfavourable free energy of folding of the unstructured linker to bring together both halves of the aptamer. The second binding event is more favourable as the two halves of the aptamer have already been brought close together. The binding of both ligands brings together the ends of the receptor that are labeled with a fluorophore-quencher pair.
Consistent with this interpretation as the length of the linker increases the more cooperative the receptor becomes.
Plaxco demonstrated these receptors using split aptamers for mercury, doxorubicin and cocaine. Ricci et al. (11) created a molecular beacon of a similar design. This beacon detected 9bp DNA sequence through two repeats of a clamp-like binding site where one half of the clamp formed Watson-Crick base pairs to the ligand and one half Hoogsteen base pairs to the same ligand. The two halves were separated by a similar disordered loop.
​
​
​
​
These cooperative receptors, and the staples of DNA origami, were the inpsiration for our repressive designs.
Molecular beacons
Broccoli
In our project, we wanted to characterise the relationship between the concentration of short RNA triggers and the translation rate of an mRNA containing our riboswitch. We estimated the translation rate using a fluorescent reporter gene (the far-red RFP variant mKate2). To quantify the concentration of the trigger strands we fused our triggers to the RNA aptamer Broccoli.
Broccoli is an RNA sequence that binds to DFHBI, a chemical similar to the GFP fluorophore. Broccoli, and its previous versions named Spinach and Spinach2, was developed by Jaffrey et al. by SELEX (12)(13).
This chemical structure of DFHBI, is non-fluorescent on its own because it can dissipate excitation via a non-radiative pathway involving cis-trans isomerisation around a single bond. When rotation around this bond is restricted, as is the case in GFP or when DFHBI is bound by Broccoli, the molecule instead decays by emitting light.
​
​
​
​
​
​
​
​
​
​
​
Broccoli is an improvement on the two spinach versions due to better folding in vivo and a reduced magnesium dependence. (14)
​
​
​
​
​
​
​
​
​
​
​
Previous methods of tagging RNA require the use of labelled oligonucleotides probes. This is difficult because of the design needed for each probe and difficulty in getting them to permeate and bind. Another method, the MS2-GFP system, involves a fusion of GFP to the MS2 phage protein. This phage protein can bind to RNA sequences in the 3’ UTR of genes. This was unlikely to have worked in our project due to the high background of unbound MS2-GFP.
Broccoli was further optimised by Jaffrey via duplication, which increased brightness and being contained within an F30 RNA scaffold, which is based upon the highly stable viral ϕ29 three-way junction RNA motif and improves folding in vivo.(15)
References
(1) Fulcrand, G., Chapagain, P., Dunlap, D. and Leng, F. (2016). Direct observation of a 91 bp LacI-mediated, negatively supercoiled DNA loop by atomic force microscope. FEBS Letters, 590(5), pp.613-618.
(2) Fulcrand, G., Dages, S., Zhi, X., Chapagain, P., Gerstman, B., Dunlap, D. and Leng, F. (2016). DNA supercoiling, a critical signal regulating the basal expression of the lac operon in Escherichia coli. Scientific Reports, 6(1).
(3) Balzer, S., Kucharova, V., Megerle, J., Lale, R., Brautaset, T. and Valla, S. (2013). A comparative analysis of the properties of regulated promoter systems commonly used for recombinant gene expression in Escherichia coli. Microbial Cell Factories, 12(1), p.26.
(4) Green, A., Kim, J., Ma, D., Silver, P., Collins, J. and Yin, P. (2017). Complex cellular logic computation using ribocomputing devices. Nature, 548(7665), pp.117-121.
(5) Espah Borujeni, A., Cetnar, D., Farasat, I., Smith, A., Lundgren, N. and Salis, H. (2017). Precise quantification of translation inhibition by mRNA structures that overlap with the ribosomal footprint in N-terminal coding sequences. Nucleic Acids Research, 45(9), pp.5437-5448.
(6) Espah Borujeni, A., Mishler, D., Wang, J., Huso, W. and Salis, H. (2015). Automated physics-based design of synthetic riboswitches from diverse RNA aptamers. Nucleic Acids Research, 44(1), pp.1-13.
(7) Green, A., Silver, P., Collins, J. and Yin, P. (2014). Toehold Switches: De-Novo-Designed Regulators of Gene Expression. Cell, 159(4), pp.925-939.
(8) Folliard, T., Mertins, B., Steel, H., Prescott, T., Newport, T., Jones, C., Wadhams, G., Bayer, T., Armitage, J., Papachristodoulou, A. and Rothschild, L. (2017). Ribo-attenuators: novel elements for reliable and modular riboswitch engineering. Scientific Reports, 7(1).
(9) Simon, A., Vallée-Bélisle, A., Ricci, F., Watkins, H. and Plaxco, K. (2014). Using the Population-Shift Mechanism to Rationally Introduce “Hill-type” Cooperativity into a Normally Non-Cooperative Receptor. Angewandte Chemie International Edition, 53(36), pp.9471-9475.
(10) Simon, A., Vallée-Bélisle, A., Ricci, F. and Plaxco, K. (2014). Intrinsic disorder as a generalizable strategy for the rational design of highly responsive, allosterically cooperative receptors. Proceedings of the National Academy of Sciences, 111(42), pp.15048-15053.
(11) Mariottini, D., Idili, A., Vallée-Bélisle, A., Plaxco, K. and Ricci, F. (2017). A DNA Nanodevice That Loads and Releases a Cargo with Hemoglobin-Like Allosteric Control and Cooperativity. Nano Letters, 17(5), pp.3225-3230.
(12) Paige, J., Wu, K. and Jaffrey, S. (2011). RNA Mimics of Green Fluorescent Protein. Science, 333(6042), pp.642-646.
(13) You, M. and Jaffrey, S. (2015). Structure and Mechanism of RNA Mimics of Green Fluorescent Protein. Annual Review of Biophysics, 44(1), pp.187-206.
(14) Filonov, G., Moon, J., Svensen, N. and Jaffrey, S. (2014). Broccoli: Rapid Selection of an RNA Mimic of Green Fluorescent Protein by Fluorescence-Based Selection and Directed Evolution. Journal of the American Chemical Society, 136(46), pp.16299-16308.
(15) Filonov, G. and Jaffrey, S. (2016). RNA Imaging with Dimeric Broccoli in Live Bacterial and Mammalian Cells. Current Protocols in Chemical Biology, pp.1-28.
(16) Butler, E., Xiong, Y., Wang, J. and Strobel, S. (2011). Structural Basis of Cooperative Ligand Binding by the Glycine Riboswitch. Chemistry & Biology, 18(3), pp.293-298.
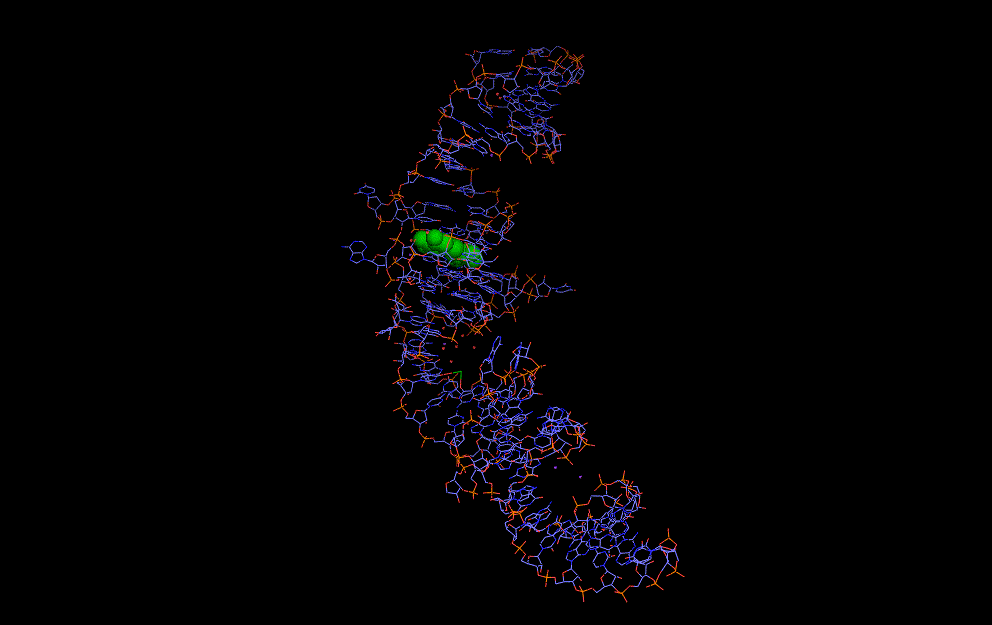
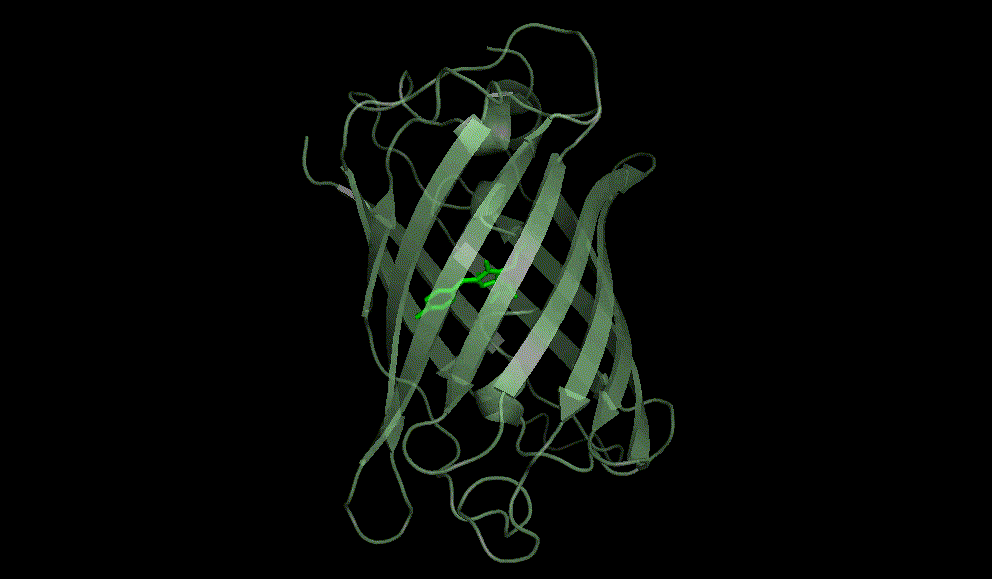
Green fluorescent protein: 1EMA
Spinach with DFHBI: 4TS0
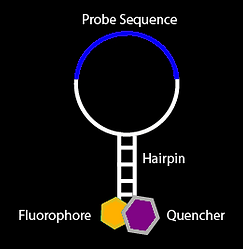
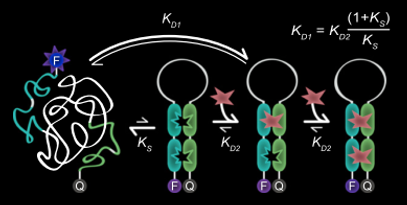
Cooperative molecular receptor adapted from Plaxco et al (10)
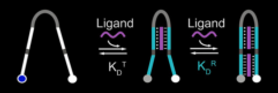
Cooperative molecular receptor adapted from Ricci et al (11)
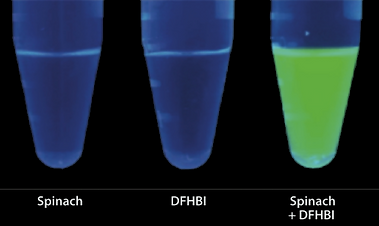
DFHBI fluorescence from You and Jaffrey (13)
Cooperative molecular beacon adapted from Plaxco et al (9)
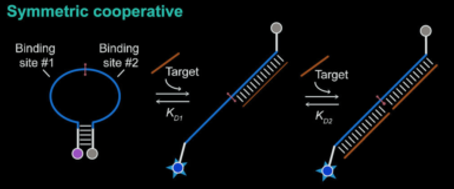